Free-Floating Planets: Journey of the Universe’s Lonely Orphans
Benj Hingston, Benjamen Smith, Justice Sparvier-Kinequon
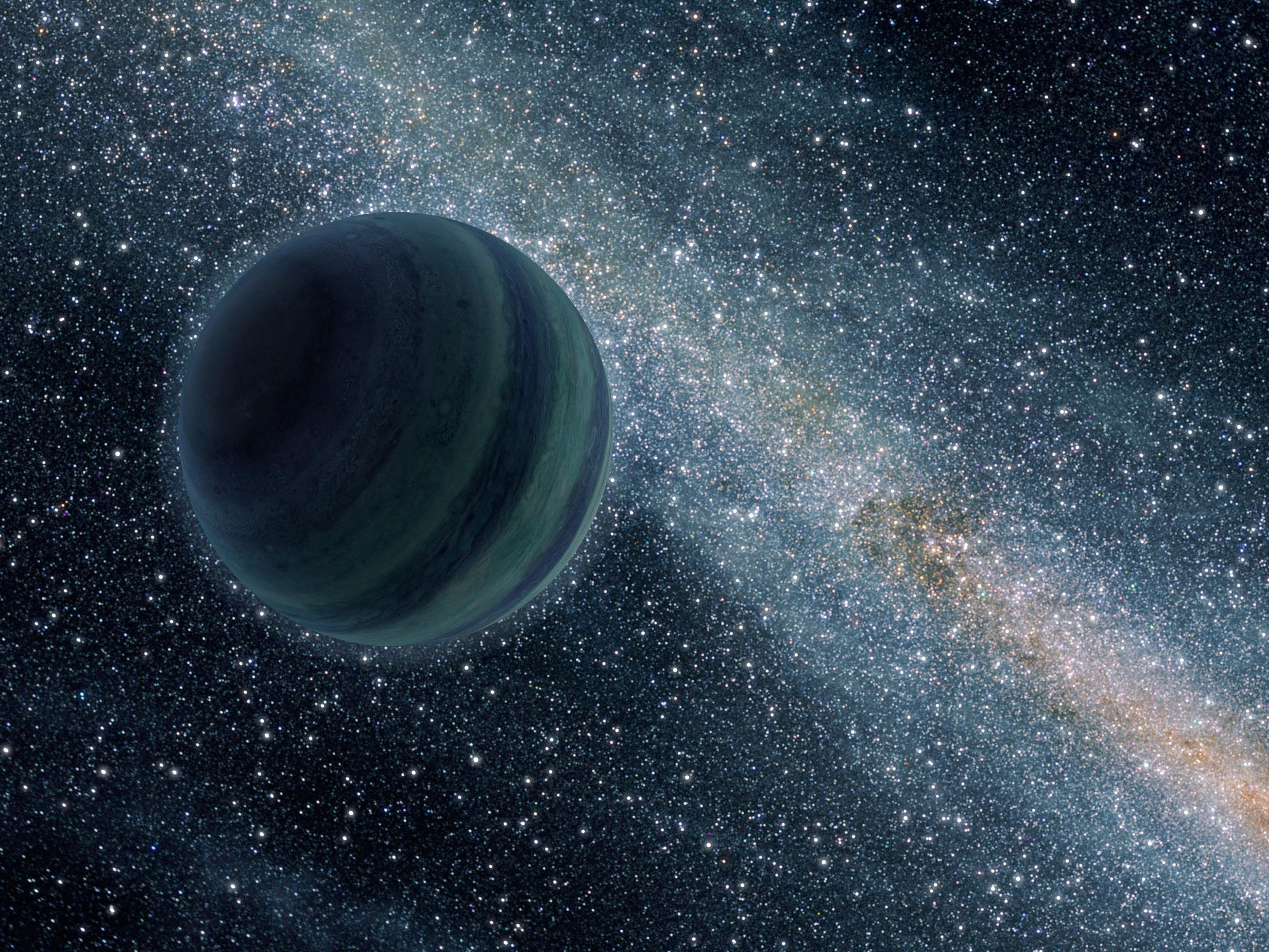
“Artist’s concept of a Free-Floating Planet” Credit JPL NASA: https://www.nasa.gov/images/content/549310main_pia14093-43_full.jpg
Abstract
In this project we will investigate rogue planets, formally known as Free-Floating Planets (FFPs). We will explore how their existence was initially supposed, and how they may be detected. Most exoplanets are detected using properties related to their host star, so detecting planets that orbit no star poses significant difficulties. Techniques like transit observation, the radial velocity method, and astrometry are all inapplicable. The primary goal of this research is to determine how rogue planet detection occurs, and establish why this method of detection is reliable. The answer is that Gravitational Microlensing is the primary method used in rogue planet detection, and its credibility arises from its derivation from Einstein’s Theory of General Relativity. Research into the origins and properties of rogue planets can tell us interesting things about the formation of our own solar system, the composition of Dark Matter in our galaxy, and the conditions necessary for life.
Rogue Planets
The Greek translation of the word “planet” is “wanderer”, which is especially fitting for rogue planets as they do not have a home; they travel aimlessly through galaxies and even “double rogue” planets wander through the interstellar medium. The double rogue status refers to the planet neither having a host star nor do they belong to a galaxy - a truly lonely place. Presently there have been very few confirmed rogue planet detections, however there are many candidates that have yet to be proven to be rogue planets as some are suspected of being brown dwarfs instead. Brown dwarfs are not planets but are protostars that do not have enough mass to maintain stable hydrogen fusion and are thus considered “failed stars”. Brown dwarfs emit limited visible light and share certain qualities with gas giants, making them difficult to distinguish as they also appear as massive dark objects.
In addition to the conventional form of a rogue planet, there are also two uniquely difficult to detect variants: hypervelocity and intergalactic. Hypervelocity rogue planets emerge when the planet ventures too close to a supermassive black hole or encounters another star and is ejected out of its orbit, reaching up to one-twentieth of the speed of light.1 Intergalactic rogue planets originate in a way similar to intergalactic stars; galactic collisions can lead to millions of planets achieving escape velocity and being ejected into intergalactic space. These variants are especially difficult to detect as their extreme qualities make them even more rare and ephemeral; detection has not been possible till recent advances in technology and precision of astronomers’ instruments.
Figure 1: “PSO J318.5-22” Credit JPL NASA:
https://exoplanets.nasa.gov/system/resources/detail_files/2085_Rouge_39x27_1200_preview.jpg
Figure 2: Comparison of relative sizes between brown dwarfs, stars and gas giant planets.
Credit Carnegie Institution for Science: https://www.eurekalert.org/multimedia/pub/web/123315_web.jpg
Simulation of Milky Way-Andromeda galactic collision
History and Discovery of the Lonely Planets
The discovery of rogue planets has happened recently in the timeline of Astronomy. The first instance was discovered by Astrophysicist Takahiro Sumi of Osaka University of Japan and Astronomer David Bennett of University of Notre Dame in a Japan-New Zealand partnership. It originally stems from a small bulge in the Milky Way galaxy in 2006-2007 and documented on 2011. They used a 1.8-meter telescope at Mt. John University Observatory in New Zealand to scan said small bulge looking for gravitational microlensing events (which will be discussed later).2 By using microlensing, they found a total of ten free floating planets around the mass size of Jupiter. Bennett guessed that planetary systems become unstable and because of that launches planets out of orbit and becomes a rogue planet. Rogue planets nowadays are quite common to detect using many methods and is suggested that there are many more (they estimate about twice as many rogue planets as stars). This estimation is based on two years of observations towards the Galactic Bulge using gravitational microlensing.3 These include planets that are also smaller than Jupiter mass size (Earth size for example). However, this includes the possibility that these stars could be very far orbits however that is unlikely the case because of the very rare cases of planetary systems with very far orbits. The history and discovery of these stars are still very new and only two planets have been actually confirmed to be a lonely planet. Thus in this century, humankind can discover new planets of this unknown topic.
Formation
There are two main mechanisms that form Free-Floating Planets, or FFPs: ejection from solar systems, and independent interstellar formation.
When gas coalesces into a star some remains as part of a circumstellar disk. Then the the gradual accumulation of dust in the disk into small rocky protoplanets creates a gravitational pull which causes gas to accumulate on the surface until a gas giant has formed. After the majority of the gas has been used up, either in the formation of gas giants or by infall into the star, rocky terrestrial planets are able to form. When a gas giant exists in an orbit very near to a smaller terrestrial planet, it is very likely that the gravitationally induced perturbation on the orbit of the terrestrial planet by the gas giant will cause the terrestrial planet to be ejected from the solar system. It is estimated that there exist 2.5 terrestrial FFPs for every star in the Milky Way. Additionally, closely orbiting systems of three or more Jupiter-like gas giants are very likely to result in one of the giants being ejected in late-stage evolution of the system. Simulations of systems with 5-100 Jupiter-likes have demonstrated that the majority of giants would eventually be ejected, leaving only a few stably bound to the remaining solar system. Comparing these suppositions, we see that terrestrial planets would typically be ejected early in the evolution of the solar system, while giants would be ejected much later. The majority of gas giant FFPs are hypothesized to have been ejected from binary star systems.4
However, not all FFPs are formed in solar systems, as some are considered to have formed independently in interstellar space. The formation of such planets is similar to the formation of stars, a cloud of gas and dust gradually accumulates together to form a unified object. If the object is too small to ignite hydrogen fusion in its core then it does not become a star. Generally, a failed star formation results in a brown dwarf, however for sufficiently low masses the resultant object can be considered a planet, so long as it falls within the proposed mass range for planets of 10^-11 to 0.012 solar masses. This mass range provides a useful criterion for distinguishing between brown dwarfs and gas giant FFPs. While both brown dwarfs and gas giant FFPs are formed by the coalescence of gases in interstellar space, FFPs are detectably smaller than brown dwarfs. Interstellar-formed FFPs are estimated to be similarly as common as brown dwarfs.
Figure 3: Artist’s depiction of a brown dwarf forming from a disk of interstellar gas.
Credit: Wikimedia Commons https://upload.wikimedia.org/wikipedia/commons/f/f1/Brown_Dwarf_11_0600.png
Detection
The detection of FFPs poses a suite of unique challenges that require special consideration not addressed by conventional exoplanet detection. Most exoplanet detection methods use observations of the host star to identify characteristics resultant of an orbiting planet. The two most successful exoplanet detection techniques are the transit method and the radial velocity method, but neither can be used for FFPs.
The transit method (Figure 4) involves making photometric observations of the star as the supposed exoplanet orbits between it and our line of sight. As the planet transits it blocks a detectable amount of the incoming light in a predictable and periodic way. The radial velocity method (Figure 5) involves using spectroscopic observations to find that a star does not merely rotate in place, but orbits around some centre of mass located very near to its centre. The centre of mass indicates that there is some other nearby object exerting a gravitational force on the star. If no luminous objects are observed coorbiting, then the likely culprit is an exoplanet. As you can see, both the transit and radial velocity methods do not involve any direct observation of the exoplanets themselves, but make inferences about them based on observations of their host star. Since FFPs do not have a host star, these methods are inapplicable.
Figure 4: Photometric light curve produced by an exoplanet transit.
Credit NASA http://www.nasa.gov/sites/default/files/images/656348main_ToV_transit_diag_full.jpg
Figure 5: The Radial Velocity Method. Credit Wikimedia Commons https://upload.wikimedia.org/wikipedia/commons/1/12/Radial_Velocity_Exoplanet_Detection.png
One method that can be used is direct imaging, which involves making direct infrared observations of an object. FFPs are easier to resolve in imaging than conventional exoplanets as there is no nearby stellar source of radiation to obscure them. However, this method requires that the subject gives off a detectable amount of infrared radiation. Most FFPs are expected to be very old, and lacking in a host star this means they have already radiated away most of their thermal energy and are consequently very dim in the infrared spectrum, making them extremely difficult to image directly.5
But there is one method that has been shown to reliably detect dim, low-mass objects in interstellar space: gravitational microlensing. General Relativity tells us that massive objects curve spacetime in a manner proportional to their mass. This curvature is what we now understand to be gravity on the cosmic scale, massive objects are drawn together as they are pulled into each other’s gravitational wells. Interestingly, Relativity also tells us that gravity can deflect light into following apparently curved paths in a way not unlike the refraction observed in optical physics. In this way, objects with a significant enough gravitational influence can act as lenses for passing light, magnifying the image of the light source. When a luminous source object and a massive lensing object are properly aligned the light from the source object is bent around the lens to create a double image of the source (Figure 6). The precise alignment of the source and lens affects the appearance of the image: if a lens is observed to be slightly to the left of the source then the right source image will be larger and brighter and the left source image will be smaller and less bright. The images will also be transformed to curve around the lensing object. If the source and lens are perfectly aligned with the line of sight then the two images wrap fully around and form an Einstein Ring (Figure 7). Since the lensing object is also acting as an optical lens the source is magnified and its apparent luminosity will increase.6
Figure 6: Simulation of Gravitational Microlensing phenomenon. As an exoplanet passes in front of a more distant star, its gravity causes the trajectory of the starlight to bend, and in some cases, results in a brief brightening of the background star as seen by a telescope. The artistic animation illustrates this effect. This phenomenon of gravitational microlensing enables scientists to search for exoplanets that are too distant and dark to detect any other way.
Credit Nasa: https://www.jpl.nasa.gov/images/kepler/20160407/kepler20160407.gif
Figure 7: A near-complete Einstein Ring.
Credit: Wikimedia Commons https://upload.wikimedia.org/wikipedia/commons/1/11/A_Horseshoe_Einstein_Ring_from_Hubble.JPG
Visual Demonstration of Gravitational Microlensing from NGP
The first confirmed observation of light following a curved path was made by a team led by Sir Arthur Eddington during the solar eclipse of 1919. By comparing the apparent locations of stars in the Hyades cluster as the eclipsed sun passed by them to their natural nighttime positions Eddington and his team were able to confirm Einstein’s prediction that light would be deflected by the gravity of a massive object like the sun. Early uses of this phenomenon involved galaxies acting as lenses, as they were the only objects massive enough to produce a detectable lensing event. This is described as Gravitational Lensing. Today, however, there exists technology sensitive enough to detect lensing events created by sub-galactic mass objects such as stars and even planets. This is Gravitational Microlensing. Microlensing is another method now used in detecting conventional exoplanets, as a planet orbiting a lensing star creates an identifiable secondary source light curve. More recently microlensing has been found to be an effective way to detect FFPs. Using large scale astrometric surveys, microlensing events can be observed wholesale over long periods of time.7 8
Once a dataset of microlensing events has been collected, there are certain indicators to be found that indicate whether a lensing event was due to an FFP or other object. Firstly, FFP’s are universally dim, so luminous lensing objects can be ruled out. Next, we know that there is a mass range for planets that distinguishes them from brown dwarfs or sub-planetary mass objects like comets. Since the magnitude of the lensing effect is related to the mass of the lensing object we can include only events with the appropriate magnifications. Additionally the duration of a lensing event is proportional to the root of the lens mass, so brief lensing events are caused by low-mass objects. This provides a secondary criterion for determining the lens object’s mass. Lastly, and most notably, are Finite Source Effects. In most lensing analyses the source object can be simplified as point-like, as the photometric light curve is similar over great enough distances and with large enough lens masses. However, if the radius of the source object projected onto the lens plane is greater than the impact parameter (the displacement of the lens from the source line of sight, perpendicular to the transverse velocity) of the lens then the source cannot be considered pointlike as the lensing effect is no longer uniform. This results is a light curve which deviates from the expected pointlike approximation, and this deviation can be used to determine the mass and transverse velocity of the lens object. The lens object’s properties can then be compared to expectations to determine if it is a candidate for an FFP.9
As with any detection method gravitational microlensing has certain advantages and disadvantages. The disadvantages derive mainly from the brevity of FFP lensing events. The duration of a lensing event is commensurate with the mass of the lensing object, and since FFPs are not gravitationally bound to any star the lensing events they induce are nonperiodic, that is each lensing event only happens once, and is very brief, with durations ranging from a couple days to mere hours. As such, it is generally impossible to perform any follow-up data collection after a lensing event has passed, and only a small amount of data can be collected from the lensing event at all. The extreme rarity and brevity of microlensing events is why large-scale sky surveys are used to attempt to make as many detections as possible across broad regions of the sky. From here the greatest advantages of microlensing derive, as the scale of observations allow the production of statistically significant samples from which estimates of planetary and FFP distributions can be made. Additionally, microlensing is uniquely specialized for detecting low-mass nonluminous objects, which makes it the best current method for detecting FFPs and similar objects.
Ramifications
Gravitational microlensing may allow spectroscopic analysis of stars that are too faint and too far away to be observed presently. Spectroscopy requires a bright enough subject to obtain spectral features distinguishable from signal noise, and so the magnification effect of microlensing may provide the necessary boost in apparent luminosity necessary to study stars in the outer limits of the galaxy. Microlensing with FFPs provides the additional advantage of having a non-luminous lensing object which will not act as an interfering light source.
One of the greatest mysteries in modern physics is that of Dark Matter. Dark matter is believed to compose the majority of matter in our universe, while “normal” matter contributes a much smaller portion. MACHO’s, or MAssive Compact Halo Objects, are one of the candidate contributors to the dark matter distribution of our universe. While present estimates suppose that MACHOs make up only a small fraction of the dark matter distribution, estimates derived from new FFP populations may affect the accepted contribution. FFPs are one of the object types that make up the MACHO category, along with brown dwarfs, black holes, and other non-luminous objects.
One of the most exciting aspects of exoplanet discoveries is the potential for finding exoplanets capable of supporting life. While there are a series of parameters necessary for life to form on a planet initially, it may turn out to be possible for life to exist even on a planet that has gone rogue. FFPs with optically thick atmospheres have the potential to retain heat energy for extremely long durations after being ejected from their original host star. Under the right temperature and pressure conditions these opaque FFPs could sustain oceans of liquid water and thus have the potential for aqueous life. Such planets would require atmospheres composed primarily of opaque enough gasses for such heat retention to occur, one of either carbon dioxide, nitrogen, methane, or ethane.10
One obstacle that remains in FFP detection is finding a method for consistently distinguishing FFPs from brown dwarfs and similar candidate objects. Presently the primary characteristic used is age, but microlensing alone is not able to determine this feature of a lensing object and so most age estimations made are circumstantial.11 Research into finding observable distinctions between FFPs and similar objects is essential.
Conclusion
In this paper we sought to explore the properties of Free-Floating Planets, how they are detected, and why we know these methods to be reliable. We found that the most reliable method involved is gravitational microlensing, a method derived from Einstein’s Theory of General Relativity. Although there are proposed to be more rogue planets than there are main sequence stars presently there have been very few confirmed rogue planet detections, a consequence of the rarity and brevity of the microlensing events we detect them by. Gravitational microlensing is extending astronomers’ reach deep into the dark crevices of our universe discovering the loneliest of planets and revolutionising our understanding of our own galaxy.
References
1 Williams, Matt “Planets Could Travel Along with Rogue ‘Hypervelocity’ Stars, Spreading Life Throughout the Universe.” Universe Today. Fraser Cain, 3 December 2014 https://www.universetoday.com/116872/planets-could-travel-along-with-rogue-hypervelocity-stars-spreading-life-throughout-the-universe/ (accessed 30 May 2018)
2 University of Notre Dame. Planets that have no stars: New class of planets discovered. https://phys.org/news/2011-05-class-planets.html (accessed 30 May 2018)
3 Unbounded or distant planetary mass population detected by gravitational microlensing. Nature vol 473, (349-352), 19 May 2011.
4 T. Barclay, E.V. Quintana, S.N. Raymon, and M.t. Penny, The Astrophysical Journal 846, 86 (2017).
5 C. Kitchin, Exoplanets: Finding, Exploring, and Understanding Alien Worlds (Springer, New York, 2012) Chap. 5-8.
6 J. Wambsganss, Nature 473, 289 (2011).
7 N. Safizadeh, N. Dalal, and K. Griest, The Astrophysical Journal 522, 512 (1999).
8 APS News, May 29,1919: Eddington Observes Solar Eclipse to Test General Relativity, <https://www.aps.org/publications/apsnews/201605/physicshistory.cfm> (Accessed June 6, 2018).
9 L. Hamolli, M. Hafizi, F. De Paolis, and A. A. Nucita, Advances in Astronomy 2015, 8 (2015).
10 V. Badesu, Acta Astronautica 69, 788 (2011).
11 Unknown, Nature 491, 498 (2012).